David Pratt
May 2001
Part 1 of 4
Contents
Part 1: The Solid Earth Hypothesis
1. The standard earth model
2. Deep drilling springs surprises
3. Mass, density, and seismic velocity (09/05)
4. Deep earthquakes
5. Geomagnetism
References
Part 2: The Hollow Earth Hypothesis
1. Early theories
2. Modern theories
3. Hollow moons
4. Feasibility — I (06/04)
5. Feasibility — II (08/05)
References
Part 3: Polar Puzzles
1. The open polar sea
2. The north pole controversy
3. Polar land cover-up?
4. Flights of fancy
5. Auroras and the poles
References
Part 4: Mythology, Paradise, and the Inner World
1. The Imperishable Sacred Land
2. Shambhala
3. A northern paradise
4. Inner kingdoms
Reference
Part 1: The Solid Earth Hypothesis
1. The standard earth model
Our direct knowledge of the earth’s interior is minuscule. The earth has a radius of about 6370 km, but the deepest scientific borehole ever drilled is only 12 km. To put this in perspective: if the earth were reduced to a tabletop globe 50 centimeters (20 inches) in diameter, the portion accessible to direct observation through the deepest borehole would be the equivalent of a very thin skin less than 1 millimeter (0.04 inch) thick. In other words, scientists have barely scratched the surface of our globe.
Nevertheless, over the past 100 years or so, geoscientists have put together a detailed picture of the earth’s interior based largely on indirect evidence — mainly the behavior of seismic waves that travel through the earth [1]. The earth’s interior is believed to consist of several concentric spheres: an outer solid crust, averaging 7 km thick beneath oceans and 35 km beneath continents; a mainly solid mantle extending to a depth of 2900 km; an outer core of liquid iron extending to a depth of 5150 km; and an inner core of solid iron, with a radius of about 1220 km.
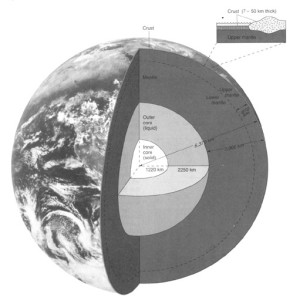
Raypaths are immensely complex; waves may undergo multiple reflections and refractions, and their paths are further complicated by the fact that lateral heterogeneity exists at every depth in the earth. This is directly indicated by the scatter in seismic-wave arrival times at all distances from the source. Seismic tomography, which seeks to image the three-dimensional structure of the earth, provides indirect evidence of lateral variations of up to 10% in seismic velocity through the crust and mantle.
Scientists cannot even begin to interpret the hundreds of thousands of seismic records without making certain basic assumptions about the earth’s interior. The main assumptions are that the earth’s interior consists entirely of solid or liquid physical matter, and that temperature, pressure, and density increase with depth. These assumptions are generally believed to be self-evident.
At several depths in the earth, there appear to be discontinuities where the velocity of seismic waves changes abruptly. Such discontinuities are often transition zones rather than sharp boundaries, and vary in depth from place to place. The dominant boundary is that between the mantle and the core. Next in order of magnitude are the crust-mantle boundary (Mohorovicic discontinuity or Moho), the inner core-outer core boundary, and the mid-mantle discontinuities at depths of 400 and 670 km. The earth’s core was ‘discovered’ in 1906, and its depth (about 2900 km) was determined by 1914. The Moho was ‘discovered’ in 1909, the inner core in 1936, and the 400- and 670-km discontinuities in the 1960s.
The thickness of the crust varies from 20 to 70 km beneath continents and from 5 to 15 km beneath oceans. As well as differing greatly in thickness, continental and oceanic crusts are said to have very different compositions: continental crust consists chiefly of granitic rock capped by sedimentary rocks, while ocean crust is believed to be composed largely of basalt and gabbro. At the crust-mantle boundary or Moho, seismic-wave velocities change abruptly, but there is no consensus on exactly why. No drillhole has yet penetrated to the Moho anywhere. The Moho varies considerably in depth, sometimes several Mohos are stacked up, and in places there is no Moho at all. Sometimes it is flat, continuous, and oblivious to faults, while in other areas it is strongly influenced by overlying geological structures and jumps from one depth to another [3].
At the two main discontinuities in the mantle, rocks are widely believed to undergo pressure transformations to denser phases. The 670-km discontinuity marks the boundary between the upper and lower mantle; seismic waves increase suddenly in speed at this depth, and earthquakes essentially cease. The mantle is thought to be composed of the dense, ultrabasic (ultramafic) rock peridotite. This is because lava sometimes contains fragments of peridotite and mountain-building processes sometimes bring up wedges of peridotite, and in both cases this rock is assumed to come from the mantle. V. Sánchez Cela disagrees and argues that many geological and geophysical phenomena can be better explained if the upper mantle is far more sialic (granitic) than currently believed [4].
The outer core is said to consist mainly of liquid iron, and the inner core of solid iron. The reasoning behind this is as follows. There are two main types of seismic body waves: P waves (compressional or longitudinal waves) and S waves (transverse or shear waves). P waves can travel through solids, liquids, and gases, while S waves can only travel through solids. Seismic waves do not reach certain areas on the opposite side of the earth from a large earthquake. P waves spread out until, at 103° of arc (11,500 km) from the epicentre, they almost entirely disappear from seismograms. At more than 142° (15,500 km) from the epicentre, they reappear. The region in between is called the P-wave shadow zone. P waves are said to be missing in the shadow zone because they are refracted by the core.
The S-wave shadow zone is larger than the P-wave shadow zones; direct S waves are not recorded in the entire region more than 103° away from the epicentre. It therefore seems that S waves do not travel through the core at all, and this is interpreted to mean that it is liquid, or at least acts like a liquid. The way P waves are refracted in the core is believed to indicate that there is a solid inner core. Although most of the earth’s iron is supposed to be concentrated in the core, it is interesting to note that in the outer zones of the earth, iron levels decrease with depth.
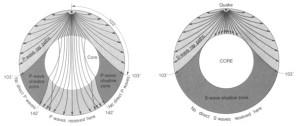
It is becoming increasingly evident that the earth model presented by the reigning theory of plate tectonics is seriously flawed [8]. The rigid lithosphere, comprising the crust and uppermost mantle, is said to be fractured into several ‘plates’ of varying sizes, which move over a relatively plastic layer of partly molten rock known as the asthenosphere (or low-velocity zone). The lithosphere is said to average about 70 km thick beneath oceans and to be 100 to 250 km thick beneath continents. A powerful challenge to this model is posed by seismic tomography, which shows that the oldest parts of the continents have deep roots extending to depths of 400 to 600 km, and that the asthenosphere is essentially absent beneath them. Seismic research shows that even under the oceans there is no continuous asthenosphere, only disconnected asthenospheric lenses.
The more we learn about the crust and uppermost mantle, the more the models presented in geological textbooks are exposed as simplistic and unrealistic. The outermost layers of the earth have a highly complex, irregular, inhomogeneous structure; they are divided by faults into a mosaic of separate, jostling blocks of different shapes and sizes, generally a few hundred kilometres across, and of varying internal structure and strength. This fact, in conjunction with the existence of deep continental roots and the absence of a global asthenosphere, means that the notion of huge rigid plates moving thousands of kilometres across the earth is simply untenable. Continents are about as mobile as a brick in a wall!
The plate-tectonic hypothesis that the present oceans have formed by seafloor spreading since the early Mesozoic (within the last 200 million years) is also becoming increasingly implausible. Numerous far older continental rocks have been discovered in the oceans, along with ‘anomalous’ crustal types intermediate between standard ‘continental’ and ‘oceanic’ crust (e.g. plateaus, ridges, and rises), and the evidence for large (now submerged) continental landmasses in the present oceans continues to mount.
2. Deep drilling springs surprises
How much faith can be put in the theories concerning the composition and density of rocks at different depths? The only place where the accuracy of scientific models can be tested directly is in the uppermost few kilometers of the crust. Although oil companies have drilled as deep as 8 km on land, they drill in sedimentary basins. The igneous and metamorphic basement, which averages 40 km thick and makes up most of the continental crust, has rarely been sampled deeper than 2 or 3 km.
The deepest borehole drilled for scientific purposes is located on the Kola Peninsula near Murmansk, Russia, in the northwestern part of the Baltic Shield. The drilling of the main borehole began in 1970, and a final depth of 12,262 metres was reached in 1994. The drilling of this and other deep and superdeep wells has produced one surprise after another, and the findings have been extremely embarrassing for earth scientists [1]. One scientist commented: ‘Every time we drill a hole we find the unexpected. That’s exciting, but disturbing.’ And a science reporter remarked: ‘Kola revealed how far from truth scientific theory can roam.’
At the Kola hole, scientists expected to find 4.7 km of metamorphosed sedimentary and volcanic rock, then a granitic layer to a depth of 7 km (the ‘Conrad discontinuity’), with a basaltic layer below it. The granite, however, appeared at 6.8 km and extends to more than 12 km; no basaltic layer was ever found! Seismic-reflection surveys, in which sound waves sent into the crust bounce back off contrasting rock types, have detected the Conrad discontinuity beneath all the continents, but the standard interpretation that it represents a change from granitic to basaltic rocks is clearly wrong. Metamorphic changes brought about by heat and pressure are now thought to be the most likely explanation.
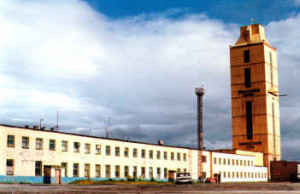
- *A nappe is a large sheet or mass of rock that has been thrust from its original position by earth movements.
Rock density is generally expected to increase with depth, as pressures rise. Results from the Kola hole indicated that densities did increase with depth initially, but at 4.5 km the drill encountered a sudden decrease in density, presumably due to increased porosity. The results also showed that increases in seismic velocity do not have to be caused by an increase in rock basicity. The Soviet Minister of Geology reported that ‘with increasing depth in the Kola hole, the expected increase in rock densities was therefore not recorded. Neither was any increase in the speed of seismic waves nor any other changes in the physical properties of the rocks detected. Thus the traditional idea that geological data obtained from the surface can be directly correlated with geological materials in the deep crust must be reexamined.’
The results of superdeep drilling show that seismic surveys of continental crust are being systematically misinterpreted. Much of the modelling of the earth’s interior depends on the interpretation of seismic records. If these interpretations are wrong at depths of only a few kilometres, how much reliance can be placed on interpretations of the earth’s structure at depths of hundreds or thousands of kilometres beneath the surface?!
Contrary to expectations, signs of rock alteration and mineralization were found as deep as 7 km in the Kola well. The hole intercepted a copper-nickel ore body almost 2 km below the level at which ore bodies were thought to disappear. In addition, hydrogen, helium, methane, and other gases, together with strongly mineralized waters were found circulating throughout the Kola hole. The presence of fractures open to fluid circulation at pressures of more than 3000 bars was entirely unexpected. The drillers at Oberpfälz discovered hot fluids in open fractures at 3.4 km. The brine was rich in potassium and twice as salty as ocean water, and its origin is a mystery.
Another surprise at the Kola hole was that lifeforms and fossils were discovered several kilometres down. Microscopic fossils were found at depths of 6.7 km. 24 species were identified among these microfossils, representing the envelopes or coverings of single-cell marine plants known as plankton. Unlike conventional shells of limestone or silica, these coverings were found to consist of carbon and nitrogen and had remained remarkably unaltered despite the high pressures and temperatures to which they had been subjected.
It is generally assumed that temperature increases with depth, reaching 1000°C at a depth of about 80 km, 4800°C at the core-mantle boundary, and 6900°C at the earth’s centre. It is certainly true that mine shafts and oil drilling operations have indicated significant increases of temperature with depth. Indeed, superdeep drilling has shown that temperature increases with depth far more rapidly than predicted. In the Kola borehole, the temperature at 10-km depth was 180°C rather than the expected 100°C. Measurements revealed significant vertical variations in temperature gradient and heat-flux density along the borehole. Overall, the rate of temperature increase rose from 11° to 24°/km down to a depth of nearly 7 km, and then started to decline. Geologists recognize that the rate of temperature increase must drop off sharply at a certain depth as otherwise the mantle would be molten below about 100 km (even at the enormous pressures assumed to exist there), whereas seismic evidence indicates that it is solid.
The oceanic crust is commonly divided into three main layers: layer 1 consists of ocean-floor sediments and averages 0.5 km in thickness; layer 2 consists largely of basalt and is 1.0 to 2.5 km thick; and layer 3 is assumed to consist of gabbro and is about 5 km thick. A drillhole in the eastern Pacific Ocean has been reoccupied four times in a 12-year span, and has now reached a total depth of 2000 m below the seafloor. Seismic evidence suggested that the boundary between layers 2 and 3 would be found at a depth of about 1700 m, but the drill went well past that depth without finding the contact between the dikes of layer 2 and the expected gabbro of layer 3. Either the seismic interpretation or the model of layer 3’s composition must be wrong [3].
As already mentioned, plate tectonics requires the crust beneath the oceans to be relatively young (no older than early Mesozoic), yet thousands of older rocks have been found in the world’s oceans, and the geological and geophysical evidence already available strongly suggests that deeper ocean drilling will uncover more ancient sediments (including further remnants of continental landmasses) beneath the basaltic layer 2 that is currently — and conveniently — labelled ‘basement’ [4]. This layer suggests that magma flooding was once ocean-wide, and studies of ocean sediments show that this activity was accompanied by progressive crustal subsidence in large sectors of the present oceans, beginning in the Jurassic.
3. Mass, density, and seismic velocity
If the earth’s interior were homogeneous, consisting of materials with the same properties throughout, seismic waves would travel in a straight line at a constant velocity. In reality, waves reach distant seismometers sooner than they would if the earth were homogeneous, and the greater the distance, the greater the acceleration. This implies that the waves arriving at the more distant stations have been travelling faster. Since seismic waves travel not only along the surface but also through the body of the earth, the earth’s curvature will clearly result in stations more distant from an earthquake focus receiving waves that have passed through greater depths in the earth. From this it is inferred that the velocity of seismic waves increases with depth, due to changes in the properties of the earth’s matter.
Seismic velocity in different media depends not just on the substance’s density but also on its elastic properties (i.e. rigidity and incompressibility). In the case of solids and liquids, for instance, there is no correlation between sound-wave velocity and density [1]. Here are some examples involving metals:
Substance | Density (g/cm³) | Velocity of longitudinal waves (km/s) |
aluminium | 2.7 | 6.42 |
zinc | 7.1 | 4.21 |
iron | 7.9 | 5.95 |
copper | 8.9 | 4.76 |
nickel | 8.9 | 6.04 |
gold | 19.7 | 3.24 |
There is a correlation between density and seismic velocity in the case of gases: velocity decreases with increasing density due to the increased number of collisions.
According to the relevant equations,* the velocity of seismic waves will become slower, the denser the rocks through which they pass, if the rocks’ elastic properties change in the same proportion as density. Since seismic waves accelerate with depth, this would imply that density decreases. However, scientists are convinced that the density of the rocks composing the earth’s interior increases with depth. To get round this problem, they simply assume that the elastic properties change at a rate that more than compensates for the increase in density. As one textbook puts it:
Since the density of the Earth increases with depth you would expect the waves to slow down with increasing depth. Why, then, do both P- and S-waves speed up as they go deeper? This can only happen because the incompressibility and rigidity of the Earth increase faster with depth than density increases. [2]
Thus geophysicists simply adjust the values for rigidity and incompressibility to fit in with their preconceptions regarding density and velocity distribution within the earth! In other words, their arguments are circular.
- *P-wave velocity = square root of [(incompressibility + 4/3rigidity) divided by density]. S-wave velocity = square root of [rigidity divided by density]. In a fluid, rigidity vanishes and S waves cannot propagate at all.
Drilling results at the Kola borehole revealed significant heterogeneity in rock composition and density, seismic velocities, and other properties. Overall, rock porosity and pressure increased with depth, while density decreased, and seismic velocities showed no distinct trend [3]. In the Oberpfälz pilot hole, too, density and seismic velocity showed no distinct trend with increasing depth [4]. Many scientists believe that at greater depths, the presumed increase in pressures and temperatures will lead to greater homogeneity and that reality will approximate more closely to current models. But this is no more than a declaration of faith.
Scientists’ conviction that density increases with depth is based on their belief that, due to the accumulating weight of the overlying rock, pressure must increase all the way to the earth’s centre where it is believed to reach 3.5 million atmospheres (on the earth’s surface the pressure is one atmosphere). They also believe that they know by how much rock density increases towards the earth’s centre. This is because they think they have accurately determined the earth’s mass (5.98 x 1024 kg) and therefore its average density (5.52 g/cm³). Since the outermost crustal rocks — the only ones that can be sampled directly — have a density of only 2.75 g/cm³, it follows that deeper layers of rock must be much denser. At the centre of the earth, density allegedly reaches 13.5 g/cm³.
Pari Spolter casts doubt on this model:
About 71% of the earth’s surface is covered by oceans at an average depth of 3795 m and mean density of 1.02 g cm-3. The average thickness of the crust is 19 km and the mean crustal density is 2.75 g cm-3. From studies of seismic wave travel time, geophysicists have outlined a layered structure in the interior of the earth. There is no accurate way currently known of estimating the density distribution from seismic data alone. To come up with a mean density of 5.5, earth models assuming progressively higher density values for the inner zones of the earth have been devised. . . .
Except for the ocean and the crust, direct measurements of the density of the inner layers of the earth are not available. This currently accepted Earth Model is inconsistent with the law of sedimentation in a centrifuge. The earth has been rotating for some 4.5 billion years. When it was first formed, the earth was in a molten state and was rotating faster than today. The highest density of matter should have migrated to the outer layers. Except for the inner core, . . . the density of the other layers of the earth should be less than 3 g cm-3.
Also, heavy elements are rare in the universe. How could so much of materials with such low stellar abundances have concentrated in the earth’s interior? [5]
The figures given for the masses and densities of all planets, stars, etc. are purely theoretical; nobody has ever placed one on a balance and weighed it! The masses of celestial bodies can be calculated from what is known as Newton’s form of Kepler’s third law. Kepler’s law states that the ratio of the cube of the mean distance (r) of each planet from the sun to the square of its period of revolution (T) is always the same number (r³/T² = constant). Newton’s version of this law assumes that r³/T² is equal to the inert mass of the body multiplied by the gravitational constant divided by 4π² (GM = 4π²r³/T²). Even if the conventional figures for the total mass and average density of the earth are correct, the prevailing earth model may still be very wrong since no one knows for certain what type of matter exists at the very centre of the earth.
The Devil’s Dictionary defines gravitation as: ‘The tendency of all bodies to approach one another with a strength proportioned to the quantity of matter they contain – the quantity of matter they contain being ascertained by the strength of their tendency to approach one another’! Such is the circular logic on which standard gravity theory is based. It should be borne in mind, however, that weight is always a relative measure, since one mass can only be weighed in relation to some other mass. The fact that observed artificial satellite speeds match predictions can be seen as evidence that the fundamentals of newtonian theory must be correct. On the other hand, the net gravitational force need not be directly proportional to inert mass, for there is plenty of evidence that characteristics such as spin and charge can modify a body’s gravitational properties [6].
4. Deep earthquakes
Most earthquakes are shallow, no deeper than 20-25 km, and occur when rocks snap and fracture under increasing stress. Earthquakes at much greater depths pose a major challenge to the standard earth model because below about 60 km, the rocks should be so hot and tightly compacted that they become ductile; instead of breaking catastrophically under stress, they should deform or flow plastically. Yet 30% of earthquakes occur at depths exceeding 70 km, and some have been recorded as far down as 700 km. Most deep-focus earthquakes occur in Benioff zones; in plate-tectonic theory these deep-rooted fault zones are labelled ‘subduction zones’, where slabs of ocean lithosphere supposedly plunge into the earth’s mantle (though there is abundant evidence contradicting this hypothesis [1]). However, some deep earthquakes have shaken Romania and the Hindu Kush where there are no ‘subduction zones’. A variety of mechanisms for deep earthquakes have been proposed, but they are all controversial [2].
The seismic radiation of deep earthquakes is similar to that of shallow earthquakes. It used to be said that deep-focus earthquakes were followed by fewer aftershocks than shallow ones, but there are indications that many of the aftershocks are simply difficult to detect, and that there is much more activity at such depths than is currently believed. The fact that deep earthquakes share many characteristics with shallow earthquakes suggests that they may be caused by similar mechanisms. However, most earth scientists are incapable of entertaining the notion that the earth could be rigid at such depths. One exception is E.A. Skobelin, who draws the logical conclusion that since deep-focus earthquakes cannot originate in plastic material but must be linked to some kind of stress in solid rock, the solid, rigid lithosphere must extend to depths of up to 700 km [3].
On 8 June 1994, one of the largest deep earthquakes of the 20th century, with a magnitude of 8.3 on the Richter scale, exploded 640 km beneath Bolivia. It caused the whole earth to ring like a bell for months on end; every 20 minutes or so, the entire planet expanded and contracted by a minute amount. A significant feature of the Bolivian earthquake was that it extended horizontally across a 30- by 50-km plane within the ‘subducting slab’. This undermines the hypothesis that such quakes are caused by olivine within the ‘cold’ centre of a slab suddenly being transformed into spinel in a runaway reaction when the temperature rises above 600°C. It also undermines the theory that gravity increases with depth; if this were true, the motion of earthquakes at such depths should be nearly vertical [4]. There appears to be something very wrong with scientific theories about what exists and what is happening deep within the earth.
The acceleration due to gravity is 9.8 m/s² at the earth’s surface and the prevailing view is that it rises to a maximum of 10.4 m/s² at the core-mantle boundary (2900 km), before falling to zero at the earth’s centre. But not all earth scientists agree. Skobelin argues that the normal, downwardly-directed gravitational force may be replaced by a reversed, upwardly-directed force at depths of 2700 to 4980 km, and that the widely-accepted figure of 3500 kilobars for the pressure at the earth’s centre, may be an order of magnitude too high [5].
Earthquakes and volcanoes tend to concentrate along certain major fault lines in the earth’s crust. The fact that heightened geological activity occurs along these ‘plate boundaries’ is sometimes hailed as one of the great successes of plate tectonics. However, it is precisely the high incidence of earthquake and volcanic activity that led geologists to label these belts as ‘plate boundaries’ in the first place! Plate tectonics sheds no light on earthquakes that happen within plates. Officer and Page state: ‘We know very little about the mechanisms involved in such intraplate earthquakes, but [they sometimes] illustrate effects that one might expect from a gigantic internal explosion, odd as such a concept may appear’ [6].
Thomas Gold has argued that, during its formation, the earth retained large quantities of hydrocarbons in its interior. He holds that various gases are sometimes released from depths of about 150 km, and when they invade the outer brittle layers of rock they weaken them by creating new fractures or reducing friction in existing faults, thereby causing or facilitating earthquakes [7]. The emission of gases (e.g. methane) from the ground is already known to cause mud volcanoes on land, circular pockmarks on the ocean floor, and ‘ice volcanoes’ or pingos on ice fields. Hydrocarbons and hydrogen are also major components of the gases emitted during major volcanic eruptions.
Eyewitness accounts provide strong evidence that gas emissions also help to cause earthquakes in general, but nowadays scientists tend to ignore these ‘subjective’ accounts in favour of ‘hard’ seismic data. Eruptions, flames, roaring and hissing noises, sulphurous odours, hazes and fogs, asphyxiation, fountains of water and mud, vigorous bubbling in bodies of water — all these are observed today in conjunction with earthquakes, just as they were in past. On the basis of such evidence, the ancients held that the movement and eruption of subterranean ‘air’ (i.e. gases) caused volcanoes if they found an outlet, and otherwise generated earthquakes. Gold argues that this mechanism could explain deep earthquakes, since he believes that the mechanism of sudden rock shear cannot operate deep in the earth’s interior. But as already noted, this belief may be wrong, and both mechanisms may apply at all depths.
5. Geomagnetism
Most earth scientists believe that, as well as having a high density, the earth’s core, unlike the mantle, must be metallic in order to generate the geomagnetic field. According to the dynamo theory, fluid motion in the earth’s outer core moves conducting material (liquid iron) across an already existing, weak magnetic field and generates an electric current. The electric current, in turn, produces a magnetic field that also interacts with the fluid motion to create a secondary magnetic field. Together, the two fields are stronger than the original and lie essentially along the earth’s rotation axis.
The main characteristics of the geomagnetic field include short-term and long-term fluctuations in intensity, reversals of polarity at irregular intervals (ranging from tens of thousands to tens of millions of years), the 11° offset between the geomagnetic axis and spin axis, and the drift of the magnetic poles around the geographic poles in an estimated period of several thousand years. Scientists assume that the dynamo theory can explain these features, though a detailed understanding is lacking. There are competing dynamo models, and a great deal of fudging is required to get the numerical models to reproduce some of the features of the actual magnetic field [1].
To explain the offset between the earth’s geomagnetic axis and the spin axis, some scientists maintain that the earth’s overall field may be a combination of a central, dynamo-created dipole field, aligned with the rotation axis, and several variable dipole fields located in the outermost portions of the core. But other scientists argue that there is no physical mechanism to generate dipoles near the core’s surface [2]. Some planets have even greater and more puzzling tilts between their magnetic and rotation axes: 46.8° in the case of Neptune, and 58.6° in the case of Uranus.
Even assuming that an outer core of liquid iron exists, there are major problems with the dynamo theory. Joseph Cater writes:
Scientists are somewhat vague as to how a magnetic field could extend 2,000 miles beyond an electric current. It requires a very powerful current to produce even relatively weak magnetic effects a very short distance above the flow. The electrical resistance of iron, at the alleged temperatures of the core, would be staggering. A steady flow of electricity requires constant potential differences. How are such potential differences produced and maintained in this hypothetical core?
The magnitude, width, and depth of such currents would have to be unbelievable to extend the magnetic field even a small fraction of the distance required, and the EMF [electromotive force] required to produce it would be even more incredible. Where could such an EMF come from? So far, scientists seem reluctant to explain this, especially since these currents are confined to a ball and would therefore follow closed paths. [3]
V.N. Larin questions whether a mechanism exists to maintain strong electric currents in the earth’s interior during its entire evolution, and argues that the very existence of active convection in the core is dubious. If convection is of thermal origin, then the source of heat in the iron core is incomprehensible. Another possibility is radioactivity, but no mechanism is known which might have segregated radioactive elements together with iron and nickel. Some scientists think that the heat source of convection may be the ongoing growth of the core. In this case, the heat would come from the potential energy of heavy particles settling in the gravity field, but this is unlikely to have lasted several billion years [4].
An alternative theory has been proposed by J.M. Herndon, who suggests that the earth’s magnetic field is largely produced by electric currents generated by a self-sustaining nuclear fission reaction in a uranium (and thorium) subcore at the centre of the earth, having a density as high as 26 g/cm³ [5]. However, the existence of such a subcore is entirely hypothetical.
Given their belief in the generation of magnetic fields by convection currents of electrically conducting liquid iron in a planet’s core, scientists were puzzled by the discovery that the Moon and Mercury had significant magnetic fields, since the Moon’s core is believed to be entirely solid and Mercury’s core nearly so. Venus is believed to have an entirely liquid core and was expected to possess a strong magnetic field, but no significant self-generated field has been detected. The magnetic fields of Jupiter and Saturn are believed to be generated by electric currents within a layer of liquid metallic hydrogen inside them, while the fields of Neptune and Uranus are thought to be produced in their superheated liquid mantles — but all this is little better than guesswork [6]. Clearly the present dynamo theory cannot explain the magnetic fields detected around some asteroids.
Alternative theories of the geomagnetic field are considered in part 2, section 4.
References
1. The standard earth model
[1] T. Lay and T.C. Wallace, Modern global seismology, San Diego, CA: Academic Press, 1995.
[2] D. McGeary and C.C. Plummer, Physical geology: Earth revealed, 3rd ed., Boston, MA: WCB, McGraw-Hill, 1998, p. 28.
[3] P. Barton, ‘Deep reflections on the Moho’, Nature, vol. 323, pp. 392-3, 1986; S. Weisburg, ‘The moho is immutable no more’, Science News, vol. 130, pp. 326-7, 1986.
[4] V. Sánchez Cela, Formation of mafic-ultramafic rocks in the crust: Need for a new upper mantle, Zaragoza: University of Zaragoza, 1999; V. Sánchez Cela, Densialite: A new upper mantle, Zaragoza: University of Zaragoza, 2000.
[5] Physical geology, p. 32.
[6] William R. Corliss (comp.), Inner earth: A search for anomalies, Glen Arm, MD: Sourcebook Project, 1991, pp. 41-3.
[7] Sue Bowler, ‘Journey to the centre of the earth’, Inside Science no. 134, New Scientist, 14 October 2000.
[8] See Sunken continents versus continental drift, and Plate tectonics: a paradigm under threat, Journal of Scientific Exploration, vol. 14, no. 3, pp. 307-52, 2000 (davidpratt.info).
2. Deep drilling springs surprises
[1] Richard A. Kerr, ‘Continental drilling heading deeper’, Science, vol. 224, pp. 1418-20,1984; Richard A. Kerr, ‘Deep holes yielding geoscience surprises’, Science, vol. 245, pp. 468-70, 1989; Richard Monastersky, ‘Inner space’, Science News, vol. 136, pp. 266-8, 1989; Taryn Toro, ‘German geology hits new depths’, New Scientist, 29 September 1990, pp. 24-5; William R. Corliss (comp.), Inner earth: A search for anomalies, Glen Arm, MD: Sourcebook Project, 1991, pp. 11-14; N.I. Pavlenkova, ‘The Kola superdeep drillhole and the nature of seismic boundaries’, Terra Nova, vol. 4, pp. 117-23, 1993; R. Emmermann and J. Lauterjung, ‘The German Continental Deep Drilling Program KTB: overview and major results’, Journal of Geophysical Research, vol. 102, pp. 18179-18201, 1997; Y.A. Popov, S.L. Pevzner, V.P. Pimenov, and R.A. Romushkevich, ‘New geothermal data from the Kola superdeep well SG-3’, Tectonophysics, vol. 306, pp. 345-66, 1999; International Continental Drilling Program (ICDP), http://icdp.gfz-potsdam.de.
[2] Kola superdeep borehole, http://icdp.gfz-potsdam.de/html/kola/wellsite.html.
[3] D. McGeary and C.C. Plummer, Physical geology: Earth revealed, 3rd ed., Boston, MA: WCB, McGraw-Hill, 1998, p. 63.
[4] J.M. Dickins, D.R. Choi, and A.N. Yeates, ‘Past distribution of oceans and continents’, in: S. Chatterjee and N. Hotton, III (eds.), New concepts in global tectonics (pp. 193-9), Lubbock, TX: Texas Tech University Press, 1992.
3. Mass, density, and seismic velocity
[1] David R. Lide (ed.), CRC handbook of chemistry and physics, Boca Raton, FL: CRC Press, 1996, pp. 14-34.
[2] A. McLeish, Geological science, Walton-on-Thames, Surrey: Thomas Nelson and Sons, 1992, p. 122.
[3] N.I. Pavlenkova, ‘The Kola superdeep drillhole and the nature of seismic boundaries’, Terra Nova, vol. 4, pp. 117-23, 1993.
[4] E. Huenges, J. Lauterjung, C. Bücker, E. Lippmann, and H. Kern, ‘Seismic velocity, density, thermal conductivity and heat production of cores from the KTB pilot hole’, Geophysical Research Letters, vol. 24, pp. 345-8, 1997.
[5] Pari Spolter, Gravitational force of the sun, Granada Hills, CA: Orb Publishing, 1993, pp. 117-9.
[6] See Gravity and antigravity, davidpratt.info.
4. Deep earthquakes
[1] Plate tectonics: a paradigm under threat, Journal of Scientific Exploration, vol. 14, no. 3, pp. 307-52, 2000 (davidpratt.info).
[2] T. Lay and T.C. Wallace, Modern global seismology, San Diego, CA: Academic Press, 1995, pp. 17-23; H. Houston, ‘Deep quakes shake up debate’, Nature, vol. 372, pp. 724-5, 1994; R.A. Kerr, ‘Bolivian quake deepens a mystery’, Science, vol. 264, p. 1659, 1994; R.A. Kerr, ‘Biggest deep quakes may need help’, Science, vol. 267, pp. 329-30, 1995; R. Monastersky, ‘Great quake in Bolivia rings earth’s bell’, Science News, vol. 145, p. 391, 1994; C. Frohlich, ‘Deep earthquakes’, Scientific American, vol. 260, pp. 32-9, 1989.
[3] E.A. Skobelin, in: C.W. Hunt (ed.), Expanding geospheres, Calgary, Alberta: Polar Publishing, 1992, pp. 41-2.
[4] M.I. Bhat, email, 2000.
[5] Expanding geospheres, pp. 35-6.
[6] Charles Officer and Jake Page, Tales of the earth: Paroxysms and perturbations of the blue planet, New York: Oxford University Press, 1993, p. 52.
[7] Thomas Gold, The deep hot biosphere, New York: Copernicus, 1999, pp. 141-63; Thomas Gold and Steven Soter, ‘The deep-earth-gas hypothesis’, Scientific American, vol. 242, pp. 130-7, 1980.
5. Geomagnetism
[1] E. Dormy, J.-P. Valet, and V. Courtillot, ‘Numerical models of the geodynamo and observational constraints’, Geochemistry, Geophysics, Geosystems, vol. 1, paper number 2000GC000062, 2000 (http://146.201.254.53/publicationsfinal/articles/2000GC000062/a2000GC000062.html).
[2] S. Bowler, ‘A simple model for planets’ magnetic fields?’, New Scientist, 16 June 1990, p. 32.
[3] Joseph H. Cater, The ultimate reality, Pomeroy, WA: Health Research, 1998, p. 163.
[4] Vladimir N. Larin, Hydridic earth, Calgary, Alberta: Polar Publishing, 1993, pp. 199-200.
[5] J.M. Herndon, ‘Substructure of the inner core of the earth’, Proc. Natl. Acad. Sci. USA, vol. 93, pp. 646-8, January 1996.
[6] Andrew Dominic Fortes, ‘Magnetic fields of the planets’, 1997, http://www.ucl.ac.uk/geolsci/edu/students/planet/student/work/magrev/magtoc.htm; W.R. Corliss (comp.), The moon and the planets, Glen Arm, MD: Sourcebook Project, 1985, pp. 185-8.
